The introduction of topology in condensed matter physics has transformed our comprehension of the electronic band structure in solid-state materials. Topological phase transitions (TPTs), representing quantum phase transitions between states with distinct topological order, can be induced by manipulating specific physical parameters. The exploration of topological states and their phase transitions presents the potential for a paradigm shift in the development of quantum devices.
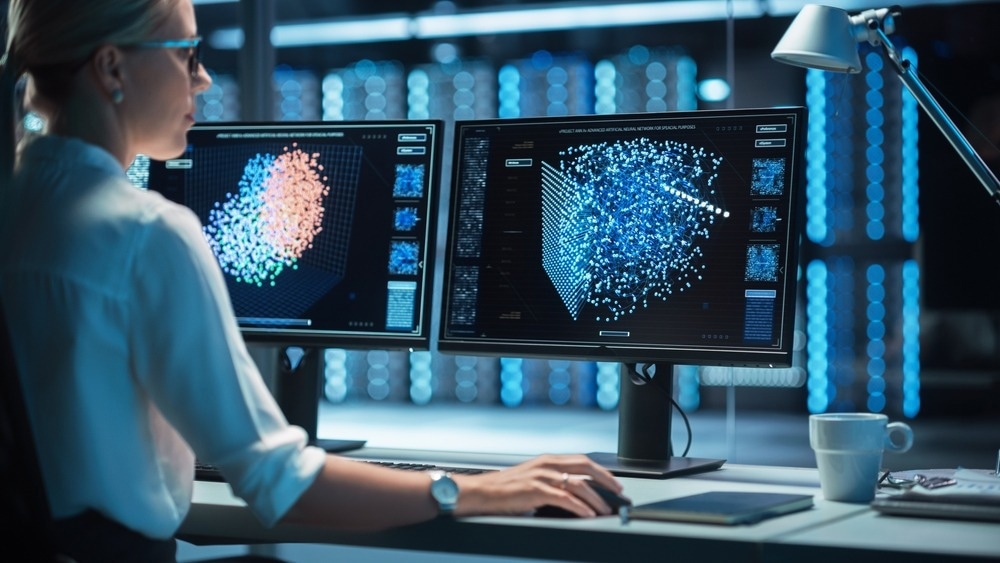
Image Credit: Gorodenkoff/Shutterstock.com
A Brief Introduction to Topological Insulators
A topological insulator is a material with a bulk electronic excitation gap resulting from the spin-orbit interaction, distinguishing it from an ordinary insulator. Researchers published an article in Physical Review B mentioning that this distinction is characterized by a Z2 topological invariant, leading to the presence of gapless electronic states on the sample boundary. In two dimensions, the topological insulator manifests as a quantum spin Hall insulator, closely related to the integer Quantum Hall state.
The spin Hall Effect has gathered significant attention in condensed matter physics, owing to its fundamental scientific significance and potential applications in semiconductor spintronics. The intrinsic spin Hall Effect, in particular, holds the promise of manipulating the intrinsic electronic properties of materials to maximize the effect.
What are the Different Topological Insulators?
Two prominent examples of topological insulators in three dimensions are Strong Topological Insulators (STIs) and Weak Topological Insulators (WTIs), as per an article published in Nature Communications.
STIs feature 2D spin-momentum-locked Dirac cones on all surfaces, prohibiting perfect backscattering but allowing general scattering off 180°. On the other hand, WTIs have surface states only on specific side surfaces. Initially considered weak, it was later discovered that the surface states of WTIs are robust due to the delocalization of surface electrons, even in cases where adjacent layers may couple, leading to a topologically trivial phase.
Weak interlayer coupling in Weak Topological Insulators (WTIs) typically results in a topological surface state with quasi-1D dispersion, preventing even general scattering off 180° and allowing for the establishment of a dissipation-less spin current, a feature not realized in Strong Topological Insulators (STIs). Despite these advantages, theoretical proposals for WTIs are scarce, and experimental investigations of topological side surfaces are challenging. Layered WTI materials are inherently cleavable only on the top surface, posing difficulties in preparing large and uniform side surfaces for observation.
Importance of Strain-Induced Topological Phase Transition?
Transitions from ordinary insulators to topological insulators (TI) can be achieved by adjusting lattice parameters and/or spin-orbit coupling through element substitution. While substitutional doping may introduce disorder, applying strain is a cleaner method to an-iso-tropically tune lattice constants in bulk or microscopic device samples.
The significance of strain-driven Topological Phase Transitions (TPTs) in solid-state systems is crucial, offering the potential for unlocking new phases of matter. Strain manipulation allows control of pseudo-magnetic fields, facilitating the development of quantum devices for applications in quantum electronics and spintronics. Identifying quantum materials sensitive to strain in their topological properties is essential to fully exploit this potential.
Latest Research Findings
Transition metal pentatellurides like ZrTe5 and HfTe5 have exhibited intriguing physical properties since the prediction of their nontrivial band topology. A comprehensive study has been published in Nature Communications focusing on the strain-driven Topological Phase Transitions from a Weak Topological Insulator (WTI) to a Strong Topological Insulator (STI) phase in high-quality HfTe5 samples. This study combines in-depth first-principles calculations with extensive quantum transport experiments.
The researchers characterized the electrical properties of as-grown HfTe5 by measuring free-standing samples. They measured the temperature dependence of the resistivity (ρxx) using the four-probe method. As the sample cooled down, ρxx slightly decreased between 180 K < T < 300 K. Further cooling led to a drastic increase in ρxx for T < 180 K until a peak in ρxx was reached at Tp - 70 K.
To investigate the strain-induced change in the electronic structure, researchers performed magneto-resistance measurements under different strains. The sample exhibited strong Shubnikov–de Haas (SdH) oscillations under all strain conditions, even for the ϵ2 case when the sample became very resistive. It was observed that the frequency of the SdH oscillations under strain ϵ2 was 1.4 ± 0.2 T, which was 16% larger than that of the unstrained ϵ0 case, 1.2 ± 0.2 T. The emergence of surface state transport aligns with the topological phase transition from a weak topological insulator to a strong topological insulator driven by strain.
By applying tensile strain along the c-axis of HfTe5, initially, the bulk gap was reduced until it was fully closed, and eventually, the band gap reopened with increasing strain. Once the gap reopened, the topological surface states (TSS) were formed.
In other words, increasing strain induced a topological phase transition from the weak topological insulator phase to a Dirac semimetal phase, and finally to a strong topological insulator phase with non-trivial surface states contributing to the electronic transport. First-principles calculations supported these findings, showing that the bulk gap is initially reduced and then increased with increasing tensile strain along the c-axis in HfTe5.
The novel research holds promise for the development of various quantum optoelectronic devices, dark matter detectors, and topologically protected devices like quantum computers. The results indicate that HfTe5 serves as an ideal prototype material for investigating strain-driven topological quantum phenomena and has the potential for application in strain-tuned topological quantum devices.
More from AZoQuantum: How the UK is Steering International Quantum Standards
References and Further Reading
Los Alamos National Laboratory, (2024). Research reveals quantum topological potential in material. [Online]
Available at: https://phys.org/news/2024-01-reveals-quantum-topological-potential-material.html
Teo, C. et. al. (2008). Surface states and topological invariants in three-dimensional topological insulators: Application to Bi 1− x Sb x. Physical Review B, 78(4), 045426. Available at: https://doi.org/10.1103/PhysRevB.78.045426
Zhang, P. et al. (2021). Observation and control of the weak topological insulator state in ZrTe5. Nat Commun 12, 406. Available at: https://doi.org/10.1038/s41467-020-20564-8
Liu, J. et al. (2024). Controllable strain-driven topological phase transition and dominant surface-state transport in HfTe5. Nat Commun 15, 332 (2024). Available at: https://doi.org/10.1038/s41467-023-44547-
Disclaimer: The views expressed here are those of the author expressed in their private capacity and do not necessarily represent the views of AZoM.com Limited T/A AZoNetwork the owner and operator of this website. This disclaimer forms part of the Terms and conditions of use of this website.